Building a brain: How does it generate its exquisite diversity of cells?
High-throughput technologies have revealed new insights into how the brain develops. But a truly comprehensive map of neurodevelopment requires further advances.
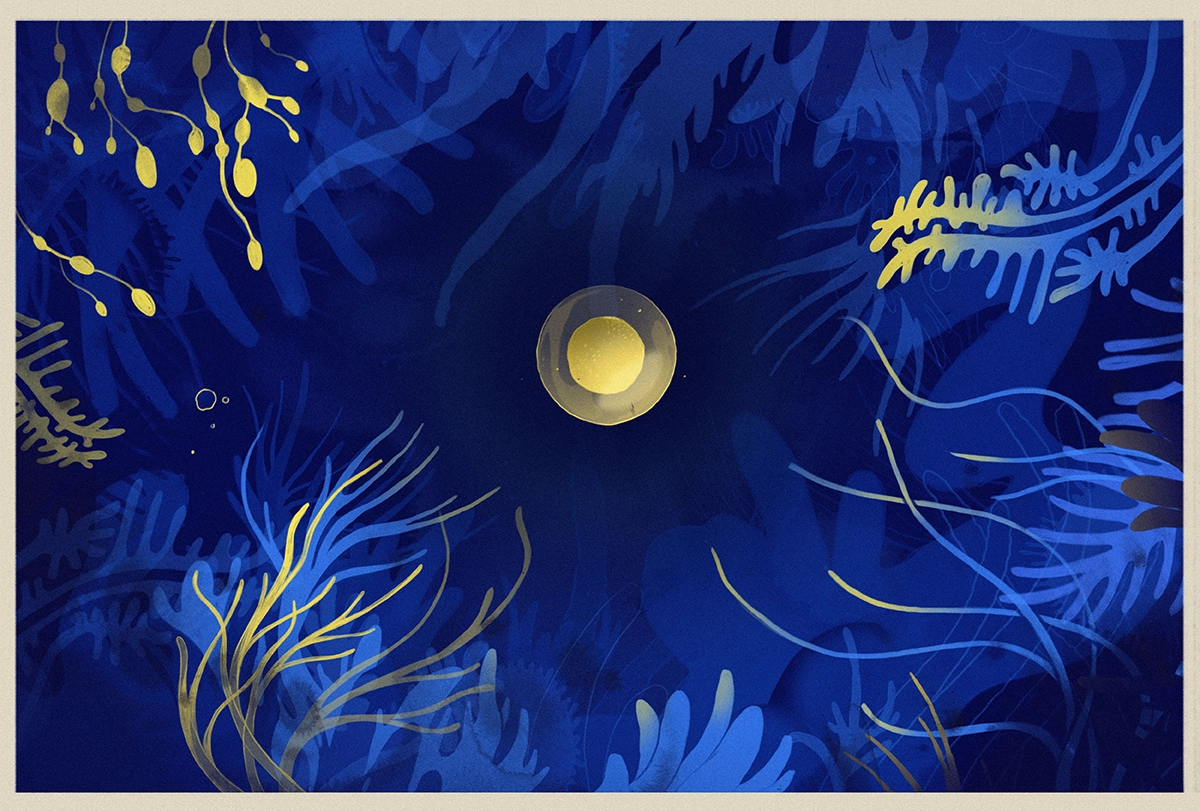
Diverse neurons and their equally diverse circuits are the foundation of the brain’s remarkable ability to process information, store memories, regulate behavior and enable conscious thought. High-throughput, single-cell profiling technologies have made it possible to classify these cells more comprehensively than ever before, offering a 360-degree view of the sheer magnitude of neural diversity in the mammalian brain. A recent effort to define the complete set of transcriptomic cell types in the adult whole mouse brain, for example, defined roughly 5,000 distinct cell types distributed across dozens of brain areas. This landmark accomplishment is a critical step toward integrating information about function and connectivity, and extending similar efforts to the adult human brain.
But this impressive gestalt conveys little, if any, information about how such diversity arises and develops in the first place. Single-cell atlases developed to date have been limited to a few points in time, focusing largely on the endpoint of neural development. How is this exquisite panoply of neurons generated and organized into precise and orderly circuits that last a lifetime? Providing the answer is the central task of developmental neuroscience. We want to understand the many transitions that unfold — where cells come from, the paths they take, and when terminal cell states emerge.
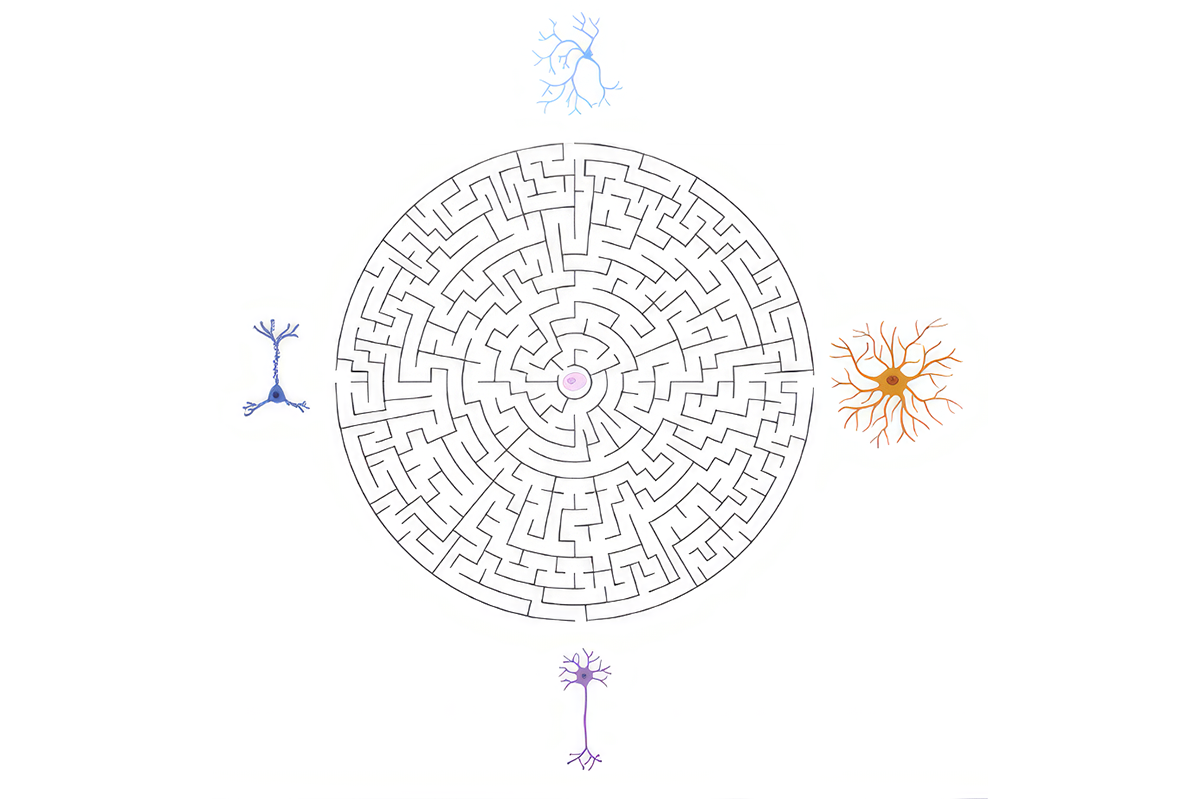
The comprehensive nature of single-cell technologies offers tremendous promise for defining cell types and reconstructing the trajectories of gene expression that underlie their differentiation. Initial efforts to apply these technologies to development, including in the prenatal human brain, hint at the insights these approaches can bring. Single-cell transcriptomics has helped map the diversity of neural progenitor cells, for example, most notably identifying progenitors that are expanded in humans, and their associated molecular adaptations. Further insights into development will require methods that reveal the specific history of every neuron type, including those that can more densely sample brain cells’ trajectories over time and novel approaches for tracking fate transitions in individual cells. These discoveries will in turn help us to understand neurodevelopmental conditions, many of which are associated with genomic variation, and neurological disorders, such as brain tumors.
S
tudies of model vertebrates, such as rodents, frogs, fish and, most recently, primates, have generated important clues into how brain cells are born. The cardinal steps underlying brain development —neurogenesis, differentiation and the formation of initial synaptic connections — are largely regulated by intrinsic mechanisms encoded in the genome and do not require input from any extrinsic sensory experience. But external stimuli can extensively refine these circuits, so that each individual brain is custom-fitted to its unique external and internal worlds. Many genetically encoded pathways of brain development are remarkably conserved across the evolutionary tree, pointing to the ancient origins of the fundamental neurodevelopmental programs. Within this largely conserved scaffold, genetic variation abounds, underpinning species-specific features.Despite these transformative discoveries, our understanding of the mechanisms that instruct neuronal diversification, maturation and wiring remains vastly incomplete. In the developing brain, neurons emerge from a limited pool of neural stem cells. Revealing the full picture of how neuronal types diversify and mature from their progenitors, we believe, will be significantly more challenging than simply classifying them. To understand the developmental history of any cell type, we need to know the evolving trajectories of the stem cells that contributed to that cell type, and to resolve the changes in molecular state, morphology, function, spatial location and connectivity along the way.
A single neural progenitor gives rise to many neuronal types through successive rounds of divisions, usually in a highly ordered, sequential manner. But neural progenitors come in diverse flavors. For example, mice have one type of radial glia, which produce one type of intermediate neuronal progenitor. Ferrets, monkeys and humans, in contrast, have three or more types of radial glia, and these may produce even more types of intermediate neuronal progenitors. We still have a long way to go to understand how these different cell types contribute to development.
C
urrent efforts to catalog brain cell types only sparsely sample the many stages of development. Building a satisfying picture of this process demands much denser sampling — by roughly two orders of magnitude, both temporally and spatially — than what is currently underway. The scale and complexity of such efforts will be huge, relying on a comprehensive sampling of the developing brain. It will require the analysis of different animals at developmental time points that span all intermediate stages of cellular differentiation, ideally using methods that preserve information about cells’ locations in the developing brain. Large-scale data integration and machine-learning approaches will also play an important role in this quest.An alternative to this dense sampling is to look for hints of a cell’s history in its epigenomic information, in particular its DNA methylation, which can harbor permanent marks of transcription factors’ past activity. Or to employ tools that individually “mark” cells with unique sequences that “track” a cell from a point of origin, where the barcode is introduced, to a terminal position in the adult brain. These powerful approaches can reveal relationships among cells long after they depart from their original progenitor cell and position. Another way is to look at the DNA mutations that accrue in cells as they divide, creating a natural ticker tape to measure distances between cells in time. The complete lineage history is thus encoded within the genomes of individual cells, although it is challenging to comprehensively read out this information with currently available technologies.
In many cases, newly born neurons migrate long distances from their site of birth to populate different brain regions. Deciphering the developmental history of neuronal types will require tracing these migratory paths and pinpointing the molecular states of precursors as they traverse them. Postnatal neuronal migration is one of the major differences between mice and humans, and an area of active investigation. Using spatially resolved genomic approaches to profile the developing brain will be an integral component of these efforts in the near future.
In the first essay in this series, author Joshua Sanes described two single-cell revolutions made possible by technological advances that revealed features of neurons that were missed or absent in previous assays or approaches. We believe that generating a comprehensive map of neurodevelopment will be a third revolution.
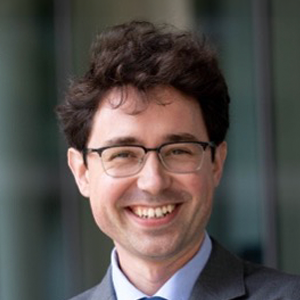
Tomasz Nowakowski
University of California, San Francisco
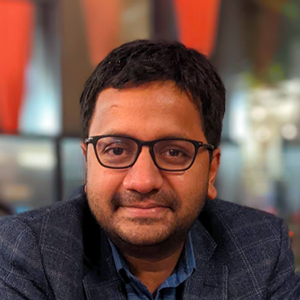
Karthik Shekhar
University of California, Berkeley
Recommended reading
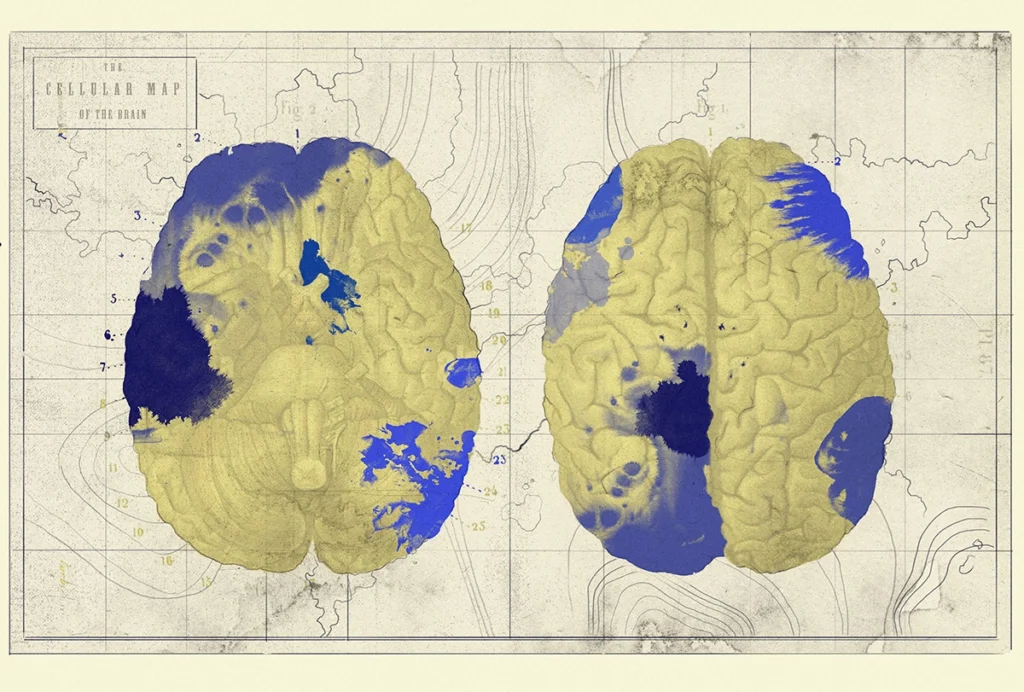
Knowledge graphs can help make sense of the flood of cell-type data
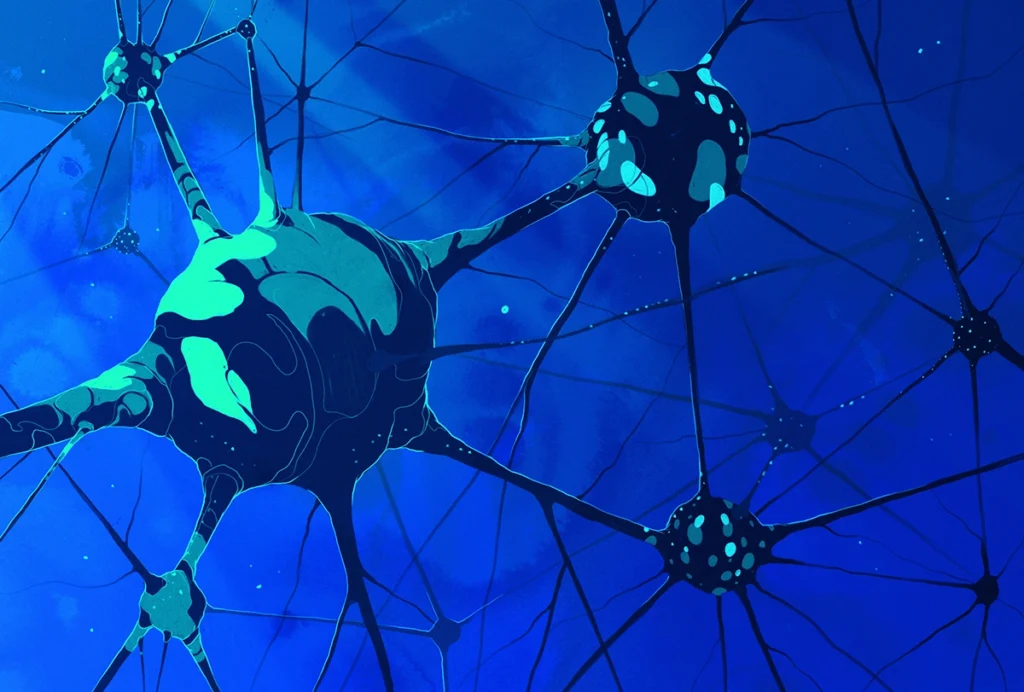
Where do cell states end and cell types begin?
Explore more from The Transmitter
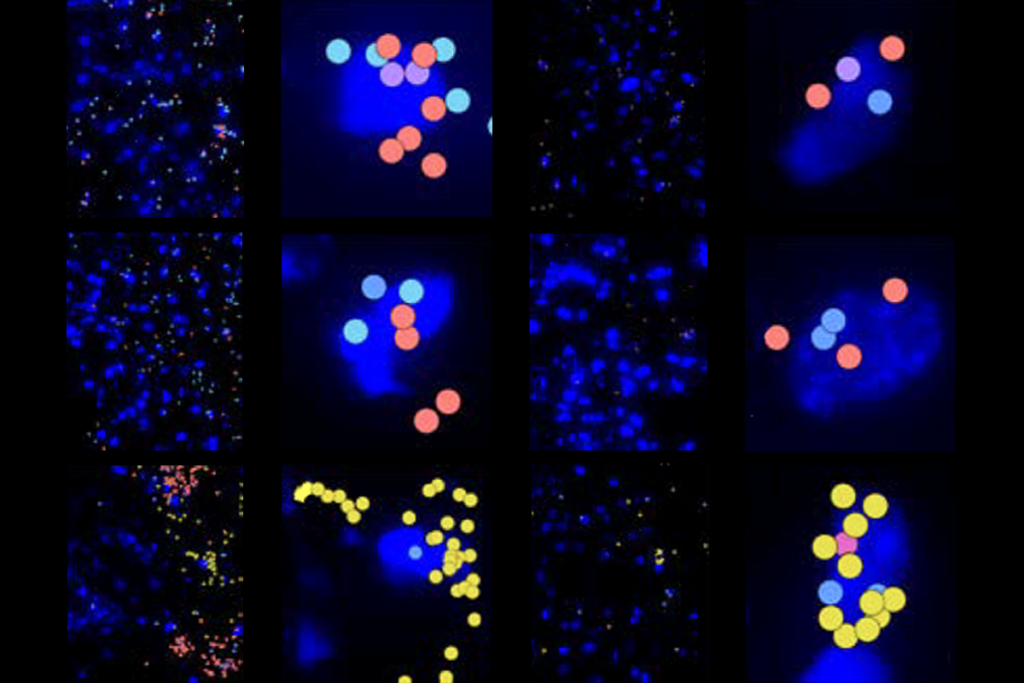
Machine learning spots neural progenitors in adult human brains
Xiao-Jing Wang outlines the future of theoretical neuroscience
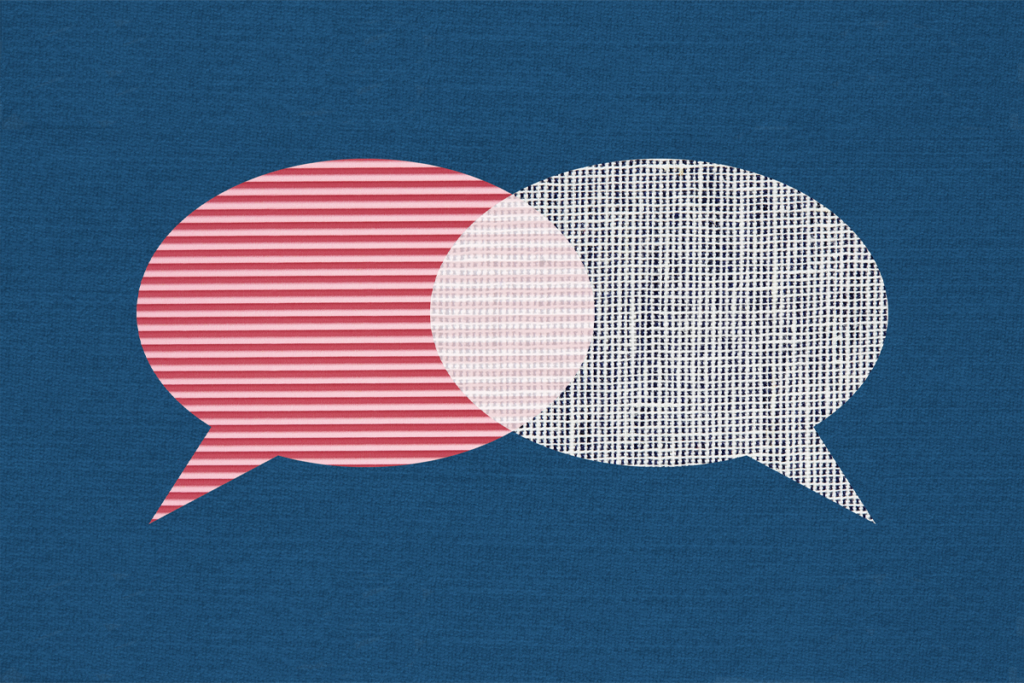